Exploring the Characterization of Nanoparticles
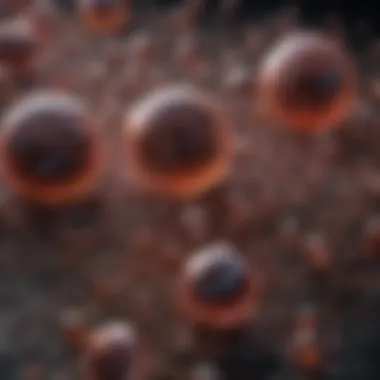
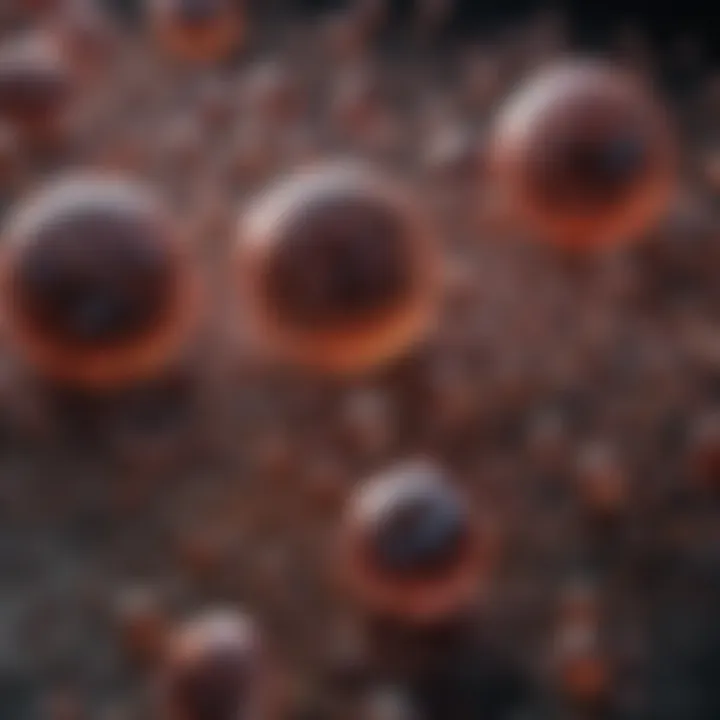
Intro
Nanoparticles represent a fascinating segment of nanotechnology, profoundly impacting diverse disciplines including medicine, electronic devices, and materials science. Their unique sizes, shapes, and properties enable innovative applications, making a thorough understanding of nanoparticle characterization imperative. Characterization encompasses numerous methodologies aimed at determining the physical, chemical, and biological attributes of nanoparticles.
This article provides a clear overview of significant characterization techniques and their relevance in various fields. It serves not just as an introduction but also as an insightful guide for students, researchers, educators, and professionals aiming to deepen their knowledge in this area. The importance of this topic cannot be overstated, given the accelerating progression of nanotechnology and its applications in real-world scenarios.
Key Findings
The exploration into nanoparticle characterization reveals several notable points:
- Diverse Techniques: A wealth of methods exists for characterizing nanoparticles, from microscopy to spectroscopy.
- Size and Shape: These two pivotal factors significantly influence the properties and behavior of nanoparticles.
- Surface Properties: Characteristics such as surface charge and functionalization play crucial roles in how nanoparticles interact with their environment.
- Biocompatibility: The interaction of nanoparticles with biological systems is vital for applications in medicine, presenting both opportunities and challenges.
Understanding nanoparticles properties is fundamental because they can behave differently from larger particles.
Major Results
Studies have shown that the size, shape, and composition of nanoparticles deeply influence their effectiveness and potential risks. For instance, smaller nanoparticles often exhibit higher reactivity and greater biological absorption rates. Similarly, spherical nanoparticles may penetrate biological membranes more effectively than larger or irregularly shaped counterparts.
Discussion of Findings
These findings suggest a critical need for standardized characterization techniques to ensure reproducibility and safety in applications. Discussions highlight discrepancies in current methodologies and the potential for improved practices that could further enhance safety.
Methodology
Research Design
Research in nanoparticle characterization often follows a multi-disciplinary approach to integrate knowledge from physics, chemistry, and biology. This design facilitates a more detailed understanding of how nanoparticles function in various environments.
Data Collection Methods
Data collection is fundamental to nanoparticle characterization. Commonly employed methods include:
- Transmission Electron Microscopy (TEM): Provides high-resolution images to study size and morphology.
- Dynamic Light Scattering (DLS): Used to determine the size distribution of nanoparticles in suspension.
- Scanning Electron Microscopy (SEM): Offers information on surface structure and topography.
- Fourier-Transform Infrared Spectroscopy (FTIR): Analyzes the chemical structure and functional groups present on nanoparticles.
Additionally, advanced techniques like X-ray diffraction and mass spectrometry contribute valuable insights into crystallinity and mass.
Prolusion to Nanoparticles
Definition and Overview
Nanoparticles can be defined as ultrafine particles with a dimension of less than 100 nanometers. At this scale, materials often exhibit properties vastly different from their bulk counterparts. Their unusual behavior is linked to the high surface area to volume ratio, which increases the influence of surface phenomena. Nanoparticles can be composed of various materials, including metals, polymers, and ceramics, and their shapes can vary from spheres and rods to complex geometries.
Such a diversity in size, shape, and material composition leads to an array of potential applications. For instance, gold nanoparticles have gained attention in medical imaging and drug delivery, while nanosilica particles are utilized to enhance the properties of plastics. A clear understanding of these definitions assists researchers in selecting appropriate materials and methods for their intended applications.
Importance in Modern Science
The significance of nanoparticles in contemporary science cannot be overstated. They lie at the intersection of physics, chemistry, and biology, driving innovations across critical sectors. Their applications in nanomedicine, such as in targeted drug delivery systems, demonstrate how nanoparticles can improve treatment efficacy by precisely delivering drugs to targeted cells while minimizing side effects. This precision medicine approach is a leap forward in healthcare.
In the realm of electronics, nanoparticles enable the development of more efficient transistors and sensors, enhancing device performance while reducing energy consumption. In environmental science, these materials show promise in purification processes, effectively removing contaminants from water.
Moreover, the ability to manipulate their properties through characterization techniques allows scientists and engineers to tailor nanoparticles for specific functions. As the field continues to evolve, ongoing research reveals new applications and deeper understandings of their behavior, thus underscoring the critical need for advancing nanoparticle characterization techniques.
Understanding nanoparticles opens doors to technological advancements that can change industries and improve lives.
Key Properties of Nanoparticles
Size and Scale Effects
The size of nanoparticles plays a pivotal role in determining their physical and chemical properties. Nanoparticles typically range from 1 to 100 nanometers in diameter. At this scale, materials can exhibit properties that are vastly different from their bulk counterparts. For example, gold nanoparticles can appear red or purple instead of metallic gold, a phenomenon linked to quantum size effects. The small size enhances phenomena such as increased reactivity and altered electrical properties.
In addition, the size distribution is equally significant. A narrow size distribution yields uniform properties, while a broad distribution can lead to inconsistencies in performance. Techniques like dynamic light scattering help in assessing size distribution, which is a vital aspect of nanoparticle characterization.
Shape and Geometry
The shape of nanoparticles can influence their interactions with surrounding environments. Various shapes such as spheres, rods, and cubes can engage differently with light, heat, and biological systems. For instance, gold nanorods demonstrate efficient photothermal properties, making them suitable for targeted drug delivery and imaging purposes. The geometry of nanoparticles dictates their surface energy and stability, impacting their aggregation behavior. This aggregation can drastically affect their efficacy in applications, especially in drug delivery systems.
Understanding shape and its influence on properties allows researchers to tailor nanoparticles for specific functionalities. Characterization methods such as electron microscopy facilitate detailed imaging to assess shape, aiding in the optimization of nanoparticles for intended applications.
Surface Area to Volume Ratio
The surface area to volume ratio is another critical property of nanoparticles. As particles decrease in size, their surface area increases relative to their volume. This heightened surface area enhances chemical reactions, making nanoparticles highly active. Higher reactivity is particularly beneficial in catalysis where increased surface area allows more interaction sites for reactants. Moreover, modifications to the surface area can be achieved through techniques like functionalization, enhancing their properties for specific applications.
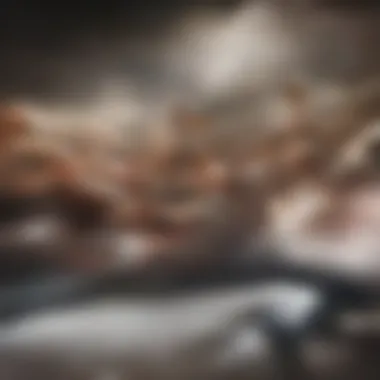
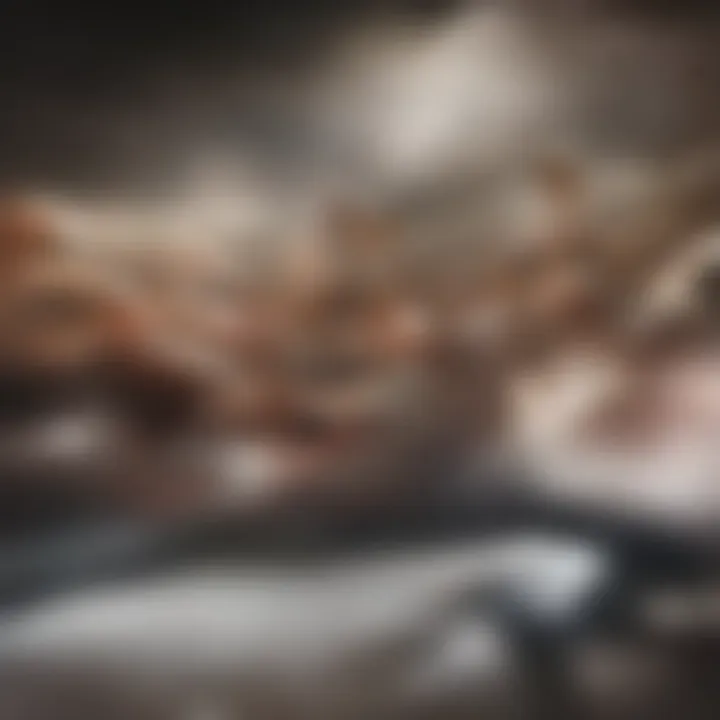
Furthermore, the surface properties dictate interactions with biological systems, affecting the toxicity and efficacy of nanoparticles in biomedical applications. The stability of nanoparticles can also be influenced by their surface coating, which can prevent aggregation and improve dispersibility. Thus, the surface area to volume ratio is crucial for the performance and application of nanoparticles in various sectors.
The key properties of nanoparticles intersect at various junctions. Understanding how these properties correlate greatly enhances the potential applications in diverse fields.
In summary, the key properties of nanoparticles are essential considerations in their characterization. The interplay of size, shape, and surface area affects their performance and suitability for specific applications. Continued research and development will inevitably unlock further potential in the use of nanoparticles.
Methods of Characterization
Microscopy Techniques
Microscopy techniques play a vital role in the direct observation of nanoparticles. They allow researchers to visualize structures at atomic or molecular levels. This means the dimensions and morphology of nanoparticles can be analyzed in detail. Several types of microscopy techniques are employed, including Transmission Electron Microscopy, Scanning Electron Microscopy, and Atomic Force Microscopy. Each has its unique advantages that facilitate in-depth inspection of nanoscale materials.
Transmission Electron Microscopy
Transmission Electron Microscopy (TEM) is a powerful imaging technique. It provides high-resolution images of nanoparticles, showcasing size and morphology effectively. One key characteristic of TEM is its ability to resolve features at atomic scales. This makes it a preferred choice in nanoparticle characterization. A unique feature is the use of a transmission beam that penetrates the sample, producing a detailed internal view of the material. However, a disadvantage is the requirement for ultrathin sample sections, which may alter the characteristics of the sample.
Scanning Electron Microscopy
Scanning Electron Microscopy (SEM) is another widely used method. It creates three-dimensional images of surface structures by scanning the surface with a focused electron beam. The key advantage of SEM is its ability to visualize surface topography. It is beneficial because of its versatility, applicable to various materials. A notable unique feature of SEM is its depth of field, which allows clear imaging even at higher magnifications. However, the need for sample coating can introduce artifacts, potentially influencing results.
Atomic Force Microscopy
Atomic Force Microscopy (AFM) is unique among microscopy methods. It measures surface forces by scanning a sharp probe over the sample surface. AFM provides detailed information about surface roughness and morphology at nanoscale. One key characteristic is its ability to measure mechanical properties in addition to imaging. This makes it advantageous for characterizing surface characteristics. However, AFM can be limited by scanning speed and may not be suitable for imaging fragile samples without inducing deformation.
Spectroscopic Techniques
Spectroscopic techniques are essential for understanding the chemical properties of nanoparticles. They provide information about the molecular composition and interactions at the nanoparticle surface. Prominent spectroscopic methods include UV-Vis Spectroscopy, FTIR Spectroscopy, and Raman Spectroscopy, each contributing uniquely to the overall understanding of nanoparticles.
UV-Vis Spectroscopy
UV-Vis Spectroscopy is a common technique for assessing electronic transitions in nanoparticles. It relies on the absorption of ultraviolet or visible light, which informs about electronic structure. The key characteristic is its ability to detect concentration changes and optical properties of materials. This technique is beneficial due to its cost-effectiveness and simplicity. However, it is limited to materials that exhibit distinct absorption in the UV-Vis range.
FTIR Spectroscopy
FTIR Spectroscopy is useful for identifying functional groups in nanoparticles. It measures the absorption of infrared light by a sample, assisting in chemical composition identification. A significant characteristic of FTIR is its ability to provide fingerprints of molecular vibrations. This makes it a beneficial choice for studying surface chemistry in-depth. However, samples may need preparation, which can sometimes alter the properties being studied.
Raman Spectroscopy
Raman Spectroscopy helps in understanding vibrational modes in molecules. By measuring inelastic scattering of monochromatic light, it provides insights into molecular structures. Its key characteristic is non-destructive analysis, allowing examination of samples without changing them. This technique stands out for its ability to work in aqueous environments. Yet, sensitivity to fluorescent backgrounds can complicate analysis, affecting data clarity.
Dynamic Light Scattering
Dynamic Light Scattering (DLS) is used for measuring the size distribution of nanoparticles in suspension. It determines the diffusion of particles through a medium by analyzing light scattering patterns. This method is known for its quick and easy measurements, making it advantageous for initial assessments of particle sizes. However, DLS can sometimes misrepresent polydisperse samples if only average sizes are reported, which could lead to incomplete interpretations.
X-ray Diffraction
X-ray Diffraction (XRD) is crucial for determining crystalline structures in nanoparticles. It provides insights on crystallinity, phase composition, and size. Its key characteristic lies in its ability to give detailed information about crystallographic directions. Thus, it’s a valuable choice for characterizing internal structures. However, XRD may not be effective for non-crystalline materials, which could limit applicability to certain nanoparticle types.
Other Techniques
Various other techniques are also significant for nanoparticle characterization. These assist in gathering comprehensive data on various parameters.
Thermogravimetric Analysis
Thermogravimetric Analysis (TGA) measures weight changes as a function of temperature, helping to understand thermal stability and composition. Its primary advantage lies in its capacity to analyze multiple thermal properties simultaneously. One unique feature is its ability to differentiate between components based on thermal degradation profiles. However, its dependence on heating rates can complicate comparisons of different materials.
Nuclear Magnetic Resonance
Nuclear Magnetic Resonance (NMR) provides information about the molecular structure and dynamics of nanoparticles. A key characteristic is its non-invasive technique for studying molecular environments. NMR is advantageous since it can provide detailed structural information. Nonetheless, the need for a strong magnetic field can limit its accessibility for some researchers.
Size Exclusion Chromatography
Size Exclusion Chromatography (SEC) separates nanoparticles based on size. It helps determine the size distribution of samples in solution. A key benefit is its gentle separation process, which helps in preserving the nanoparticles' integrity. Its unique feature is the straightforward interpretation of elution profiles. However, SEC may be less effective for particles with very similar sizes, complicating accurate analysis.
Importance of Size and Shape Control
The size and shape of nanoparticles are central to their characteristics and functionality. Understanding these factors is essential for their applications in fields like nanomedicine, electronics, and environmental science. Effective control over size and shape can lead to significant enhancements in performance, enabling the design of nanoparticles that are optimized for specific tasks.
The implications of nanoparticle size are numerous. Smaller particles generally exhibit increased reactivity due to a larger surface area relative to their volume. This property makes them particularly useful in catalytic applications. Conversely, larger nanoparticles may provide stability in certain contexts but may not be as effective for targeted drug delivery in medicine.
Shape is equally important; it influences the way nanoparticles interact with their environment, affecting their dispersion in solvents, biological interactions, and even optical properties. In many cases, different shapes can showcase varying efficacy in drug delivery or targeting cancer cells.
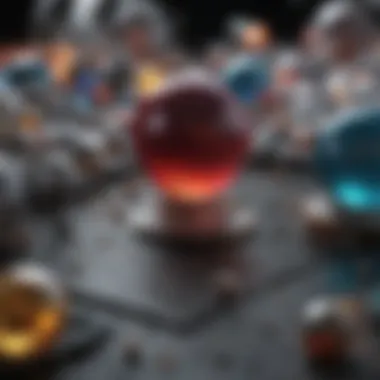
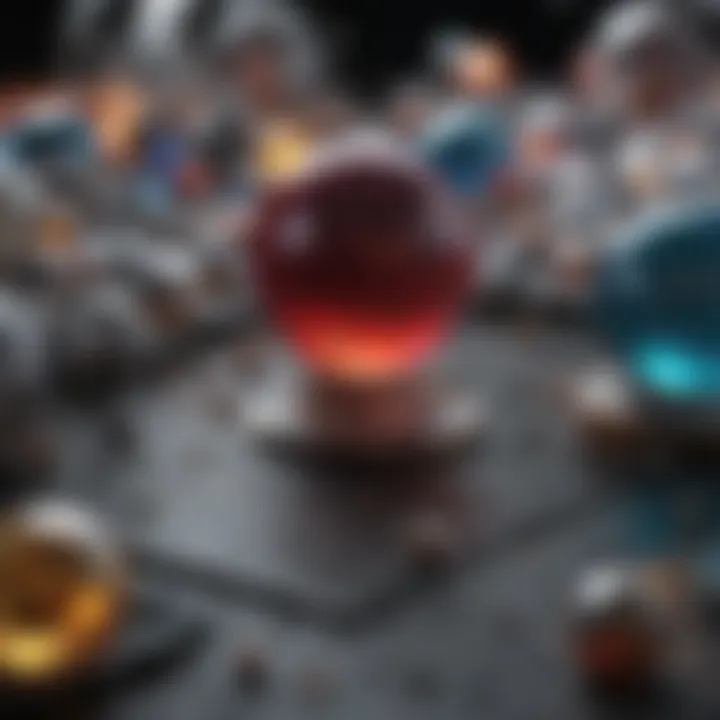
Synthesis Methods Impacting Characteristics
Top-Down Approaches
Top-Down Approaches refer to techniques that begin with bulk materials and break them down into nanoscale particles. This method includes processes like milling, lithography, and etching. One of the key characteristics of Top-Down Approaches is their ability to produce well-defined sizes and shapes reliably, which makes them a beneficial choice for many applications.
A unique feature of this method lies in its scalability. It is often easier to scale up Top-Down processes, making them suitable for industrial production. However, a potential disadvantage might be the introduction of defects and impurities during the mechanical processes. This could affect the nanoparticles’ overall functionality in various applications.
Bottom-Up Approaches
In contrast, Bottom-Up Approaches involve assembling nanoparticles from molecular or atomic components. These include chemical vapor deposition and sol-gel processes. The main characteristic of Bottom-Up Approaches is their ability to create nanoparticles with high purity and uniformity. This makes them particularly appealing in applications where consistency is critical, like drug formulations.
A unique trait of Bottom-Up techniques is their versatility in producing a wide variety of nanoparticle compositions and morphologies. However, challenges with scaling and controlling particle size can arise, which need careful consideration during the synthesis process.
Influence on Physical and Chemical Properties
The influence of size and shape on nanoparticles extends beyond mere aesthetics; it plays a crucial role in determining their physical and chemical properties. These properties include reactivity, conductivity, strength, and thermal stability. For instance, gold nanoparticles exhibit different colors depending on their size due to localized surface plasmon resonance phenomena. This effect can be leveraged in applications such as sensing and imaging.
In addition, the chemical reactivity of nanoparticles can be tailored by their size and shape, allowing for specific interactions with other molecules. Understanding these influences is paramount for the effective application of nanoparticles in various sectors, as they help dictate how nanoparticles behave in biological systems and environments.
Surface Properties and Modifications
The surface properties and modifications of nanoparticles are crucial for their application in various fields. These characteristics can greatly influence their interactions with biological systems, other materials, and their overall functionality in technological applications. Understanding these properties allows researchers to tailor nanoparticles for specific uses, improving effectiveness and safety.
Surface Chemistry
Surface chemistry refers to the chemical interactions occurring at the surface of nanoparticles. The surface atoms can behave differently than those inside the bulk of the material. This difference can affect reactivity, stability, and environmental interactions.
Surface functional groups can be introduced or modified to enhance the desired characteristics of nanoparticles. These modifications can improve solubility, promote stability, and enable specific interactions with biological molecules. For instance, the addition of carboxyl or amine groups can enhance biocompatibility, making nanoparticles suitable for drug delivery systems.
Additionally, the choice of stabilizing agents or surfactants utilized during nanoparticle synthesis can alter the surface properties significantly. Proper selection is key to achieving the intended application. A well-modified surface can also prevent aggregation, which is often a challenge in nanoparticle applications.
Coating and Functionalization
Coating and functionalization are pivotal to the practical applications of nanoparticles. Coatings can provide a protective layer that can minimize oxidation and degradation. This is particularly important in sensitive environments where nanoparticles may be exposed to reactive agents.
Functionalization deals with attaching specific molecules or groups to the nanoparticle surface that impart specific functions. For example, coating gold nanoparticles with polyethylene glycol enhances their stability and biocompatibility, making them favorable for use in medical applications. Common coatings include polymers, biomolecules, and silanes, each offering unique benefits depending on the targeted application.
Trade-offs should be considered; specific coatings can affect both the performance and efficacy of the nanoparticles over time. Thus, a careful evaluation is necessary during the design phase.
Stability and Aggregation
Stability and aggregation are paramount in determining the applicability of nanoparticles. Aggregation can occur due to van der Waals forces or other environmental factors, leading to loss of functionality and performance.
To avoid this, surface modifications are utilized to enhance dispersibility in desired mediums. These modifications can include adjusting the surface charge or using steric stabilizers that create a repulsive barrier, thus preventing close contact between nanoparticles.
It is essential to measure the stability through dynamic light scattering and other techniques to ascertain the degree of aggregation over time. An effective stability strategy will ensure that the nanoparticles maintain their desired characteristics throughout their intended lifecycle.
"Effective surface modification not only addresses stability but can tailor nanoparticles for specific interactions and purposes."
Through comprehensive understanding and manipulation of surface properties and modifications, researchers can significantly enhance the functionality of nanoparticles. This can lead to novel applications in areas such as nanomedicine, environmental remediation, and electronics. Careful consideration of all aspects involved in surface modifications ensures better performance and broader applicability in various fields.
Biological Characterization
Biological characterization is vital in understanding how nanoparticles interact with living systems. This aspect focuses on their behavior within biomedical environments, including their distribution, cellular uptake, and consequent effects on biological activities. As nanoparticles become increasingly utilized in medical applications, comprehending their biological interaction helps ensure safety, efficacy, and functionality in therapies and diagnostics.
Interaction with Biological Systems
The interaction of nanoparticles with biological systems can be complex. These nanoscale materials may present unique properties not found in their bulk counterparts. As nanoparticles enter biological systems, their size, shape, and surface chemistry play essential roles in determining how they interact with cells, tissues, and organs. For instance, nanoparticles can be targeted to specific cell types by modifying their surface characteristics.
- Size Considerations: Smaller nanoparticles often penetrate biological membranes more easily than larger ones. This factor is particularly important in drug delivery, where efficient cellular uptake enhances therapeutic outcomes.
- Surface Chemistry: The functionalization of nanoparticle surfaces with specific ligands can facilitate or inhibit interactions with biological entities. For example, functionalization can help enhance the selectivity of nanoparticles towards cancer cells, improving targeting capabilities.
- Shape and Geometry: The geometry of nanoparticles affects how they are perceived by the immune system. Certain shapes may be more readily recognized and cleared by immune cells, while others can evade detection, leading to prolonged circulation times in the bloodstream.
Understanding these interactions is essential for developing safer and more effective nanomedicine applications.
"The potential for nanoparticles to revolutionize medical treatments hinges on our comprehensive understanding of their biological interactions."
Toxicological Assessment
Toxicological assessment is a critical area of focus within biological characterization. As nanoparticles are increasingly integrated into consumer products, pharmaceuticals, and medical devices, evaluating their potential toxicity becomes crucial. The toxicity of nanoparticles can arise from several factors, including their chemical composition, size, shape, surface chemistry, and the dose administered.
There are several core aspects to consider in toxicological assessments:
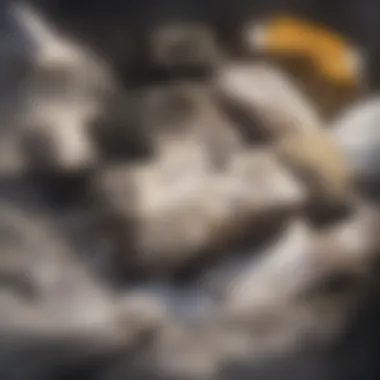
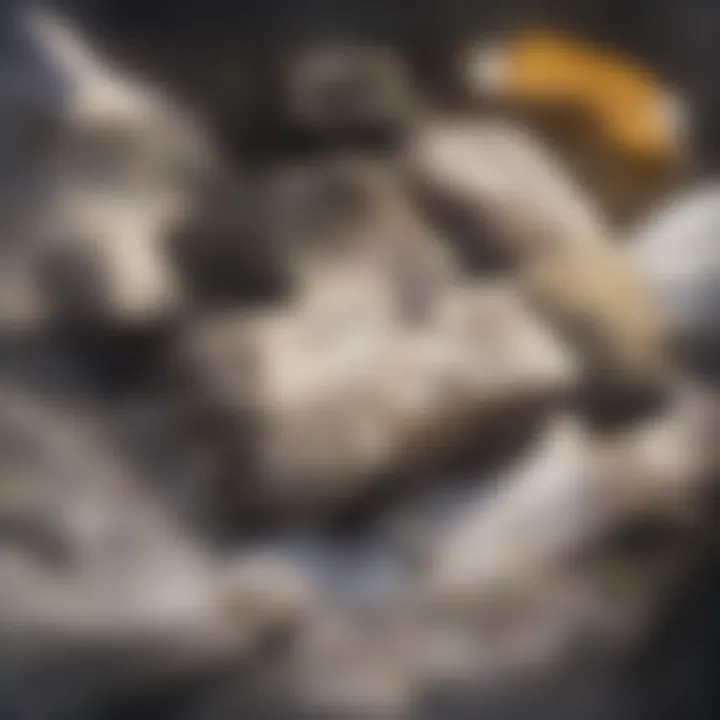
- Cell Viability: Tests often involve evaluating the impact of nanoparticles on living cells, examining whether they induce cytotoxicity at various concentrations.
- Inflammatory Responses: Assessing how nanoparticles influence inflammatory markers is important. An increased inflammatory response can signal potential adverse biological effects.
- Genotoxicity: Understanding whether nanoparticles can cause DNA damage is vital. Genotoxic effects may lead to mutations or cancer, raising significant public health concerns.
- Chronic Exposure Studies: Long-term exposure assessments are necessary to identify potential cumulative effects resulting from protracted interactions with nanoparticles.
Overall, toxicological assessments provide essential insights into the risks associated with nanoparticle exposure, helping inform regulatory guidelines and safety assessments within the realms of healthcare and environmental impact.
Applications of Characterization Techniques
Characterization techniques for nanoparticles are vital to translating theoretical findings into practical applications. The insights derived from these techniques impact various sectors, including medicine, electronics, and environmental science. Emphasizing the ability to analyze and manipulate properties at the nanoscale can open new avenues for innovation.
The applications of characterization techniques extend beyond simple measurement. They help confirm the effectiveness and safety of nanomaterials in real-world settings. Understanding the interplay between the structure and function of nanoparticles is critical for engineers and scientists alike. In this section, we explore the specific applications that benefit from these characterization techniques.
Nanomedicine
Nanomedicine employs nanoparticles to enhance drug delivery systems. Characterization techniques help in determining drug release profiles and interaction dynamics within biological systems. For example, techniques such as Dynamic Light Scattering provide size distribution and help in determining the optimal size of nanoparticles for cellular uptake.
Moreover, spectroscopy techniques give essential insights into the interactions between nanoparticles and biological targets. This understanding is crucial in designing novel therapeutic agents that maximize efficacy while minimizing toxicity. The ongoing development of targeted drug delivery systems depends heavily on rigorous characterization methods.
Key Considerations for Nanomedicine:
- Biocompatibility: Understanding how nanoparticles interact with living systems is essential.
- Controlled Release: Characterization helps achieve desired drug release rates, improving treatment outcomes.
- Imaging: Techniques like Raman Spectroscopy aid in visualizing drug distribution at the cellular level.
Electronics and Photonics
Nanoparticles also play a pivotal role in electronics and photonics. Characterization techniques inform the design and application of nanomaterials in semiconductors and photonic devices. For instance, Transmission Electron Microscopy allows for detailed imaging of nanoparticle shape and crystallinity, which are fundamental for optimizing electronic properties.
In photonics, the size and shape of nanoparticles can profoundly influence light absorption and scattering. Techniques such as UV-Vis Spectroscopy provide insights into these interactions, allowing for the engineering of materials that can manipulate light effectively. With nanostructured materials, new functionalities, like enhanced photovoltaic efficiency or novel optical sensors, become feasible.
Benefits in Electronics and Photonics:
- Enhanced Performance: Carefully characterized nanoparticles lead to better device efficiency.
- Customization: Understanding characteristics allows for tailoring materials to specific applications.
- Minimized Losses: Effective characterization reduces energy losses in electronic components.
Environmental Applications
Characterization techniques also serve critical functions in environmental applications. They are essential in assessing the impact of nanoparticles on ecosystems. Characterization helps identify the partitioning behaviors of nanoparticles in environmental matrices, their stability, and potential accumulation in biota.
For instance, X-ray Diffraction provides information on the crystalline structure of nanoparticles, which affects their mobility in environments like soil and water. Additionally, techniques like Thermogravimetric Analysis can estimate the stability of nanoparticles under different environmental conditions.
Considerations for Environmental Applications:
- Impact Assessment: Understanding how nanoparticles behave in the environment is crucial for safety evaluations.
- Remediation Solutions: Characterization informs the design of nanoparticles used in environmental clean-up efforts.
- Legislative Compliance: Ensuring that materials meet regulatory safety standards heavily relies on accurate characterization.
"Characterization techniques enable a deep understanding of how nanoparticles function in various applications, ensuring safety and efficacy across fields."
In summary, the applications of characterization techniques in nanotechnology are as broad as they are significant. Whether in healthcare, electronics, or environmental science, the insight gained through these methods is indispensable for innovation and safe implementation.
Future Perspectives
The field of nanoparticle characterization is rapidly evolving, fueled by advancements in technology and an increasing understanding of nanomaterials. As researchers and industries continue to explore the potential of nanoparticles in various applications, the future perspectives of characterization techniques hold great significance. This section highlights the emerging methods, expected trends, and the benefits they will bring to the field.
Innovative Characterization Techniques
Recent developments have led to the inception of innovative characterization techniques that promise to enhance the accuracy and efficiency of nanoparticle analysis. For instance, single-particle ICP-MS (Inductively Coupled Plasma Mass Spectrometry) is emerging as a powerful tool for characterizing nanoparticles in complex matrices. This technique allows for the detection of individual nanoparticles, providing critical information about their size, composition, and concentration, ultimately leading to better understanding of their behavior in various environments.
Moreover, nano-FTIR microscopy combines element-specific identification with nanoscale imaging, thereby allowing scientists to investigate the chemical composition of nanoparticles at unprecedented spatial resolution. Another interesting technique is correlative microscopy, which merges different microscopy methods to provide comprehensive data about nanoparticles, enabling detailed imaging alongside chemical analysis.
Challenges in Characterization
Despite the exciting advancements in characterization techniques, several challenges persist that could impede further progress. One of the primary difficulties is the inherent complexity of nanoparticles. Their diverse behaviors in different environments can lead to inconsistent results across methodologies. Reproducibility is a major concern, as varying preparation methods or environmental conditions can yield significantly different outcomes.
Additionally, many characterization techniques require specialized equipment and expertise, which can hinder access, especially in developing regions. The high costs involved in acquiring and maintaining cutting-edge equipment limit opportunities for widespread adoption.
Another challenge lies in the interpretation of results. As nanoparticle characterization becomes more sophisticated, the data produced can be overwhelming. Researchers must be equipped with the tools and knowledge to interpret and make sense of substantial data sets.
Overall, the future of nanoparticle characterization is filled with potential but requires careful navigation of these challenges to fully realize the benefits of innovative techniques for high-precision analysis and application.
The End
Summary of Key Insights
- Diverse Techniques: The range of methodologies, from microscopy to dynamic light scattering, offers comprehensive tools for effective nanoparticle analysis.
- Impact of Size and Shape: Understanding the effects of nanoparticle dimensions and shapes is fundamental to optimizing their functionality in diverse applications.
- Surface Modifications: The ability to modify surface properties significantly enhances the stability and interaction capabilities of nanoparticles within various environments.
These points underscore the necessity to employ vigorous characterization methods to fully harness the potential of nanoparticles in real-world applications.
Significance of Ongoing Research
Ongoing research in the field of nanoparticle characterization is indispensable for several reasons. Firstly, as the technology evolves, so does the complexity of the materials involved. Continuous exploration enables the development of more refined techniques, staying abreast of advancements in nanotechnology.
Secondly, understanding biological interactions with nanoparticles will be a key factor in ensuring safety and efficacy in medical applications. An investigation into potential toxicological effects remains critical, emphasizing the need for thorough characterization processes. By tackling challenges and innovating in this space, researchers can unlock new possibilities for applying nanoparticles in medicine and other domains, further advancing science with robust, reliable data.
"The future of nanotechnology heavily relies on our capacity to characterize materials accurately and adapt accordingly."