Electroformation: An In-Depth Exploration of Techniques and Applications
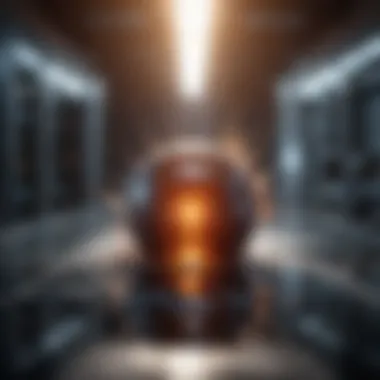
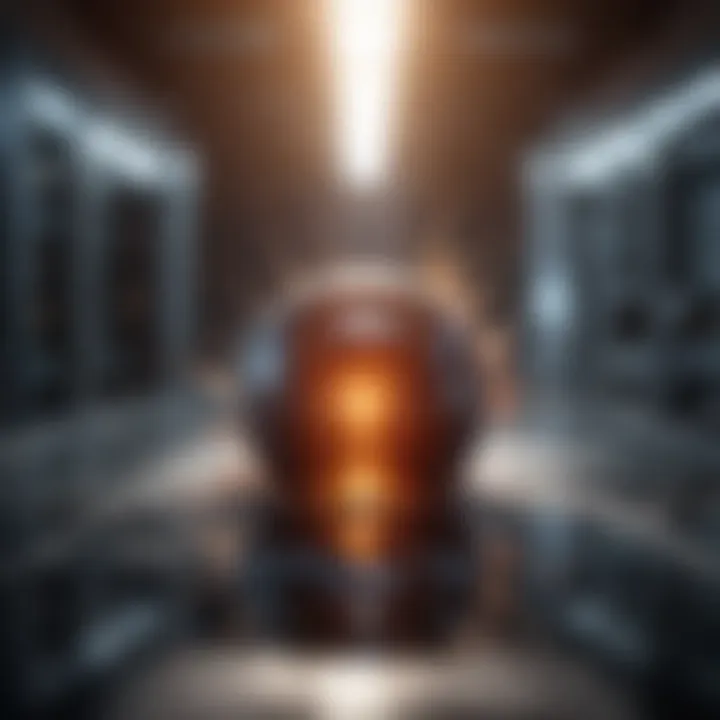
Intro
Electroformation is a nuanced process that plays a crucial role in the realm of advanced materials and nanotechnology. The practice revolves around the careful manipulation of electrical fields to create various structures at the nanoscale. It encompasses a combination of chemistry, physics, and engineering principles, all aimed at producing materials with specific properties. Understanding electroformation involves more than just its mechanisms; it also requires an exploration of its applications and implications across different fields.
In recent years, electroformation has gained traction in various industries, including biomedical engineering, electronics, and even energy storage. This growing interest highlights the need for a comprehensive exploration of the topic, ranging from theoretical foundations to practical applications. The significance of electroformation is not just in its ability to produce innovative materials but also in enhancing existing technologies and creating new opportunities.
This article will guide the reader through the fundamental aspects of electroformation, including its methodologies, the principles behind the process, its multitude of applications, and the challenges facing its broader implementation. With this detailed understanding, scholars, researchers, and industry professionals can appreciate the transformative potential of this important fabrication technique.
Understanding Electroformation
Electroformation represents a significant intersection of chemistry and engineering. It is crucial in manufacturing nanostructures and advanced materials, making it an essential topic for researchers and practitioners in materials science. The process leverages electrochemical principles to form complex structures at the nanoscale, providing solutions that are often not attainable through traditional methods. Understanding electroformation is vital as it opens avenues for innovation and application across various domains, including nanotechnology, biomedical engineering, and energy storage.
Definition of Electroformation
Electroformation is a process that involves the formation of materials through electrochemical reactions. During this process, controlled electric fields induce the growth of structures, either by depositing materials at an electrode or through molecular alterations in a solution. This technique is pivotal for creating thin films, nanostructures, and other advanced materials that have unique properties and functionalities.
Historical Background
The development of electroformation has a rich history that underscores its importance in modern science.
Early Research
Early research in this field focused on understanding how electric currents could manipulate materials at the microscopic level. One of the key aspects of early efforts was the exploration of electrodeposition and its potential to produce uniform coatings and structures. This foundational work contributed to the broader knowledge of electrochemistry and established a basis for future technical advancements. The studies were largely experimental, often requiring trial and error to refine techniques and outcomes. This made early research a necessary yet complex journey towards reliable electrochemical processes.
- Key Characteristic:
Early experiments often faced limitations in scalability and reproducibility. This made it a challenging endeavor, but it also provided significant insights that shaped the direction of the field.
Key Milestones in Development
Over the years, electroformation has seen crucial milestones that have advanced its practical applications. Notably, the introduction of new materials and process automation has drastically improved the effectiveness of electroformation techniques. The development of better conductive inks and electrolytic solutions enabled researchers to explore a wider range of materials and configurations.
- Key Characteristic:
The refinement of electrochemical cells and electrode design has played a pivotal role. Continuous improvements in these areas led to enhanced precision and control in forming structures.
In summary, past research and milestones in electroformation lay the groundwork for today's innovations. By understanding its evolution, we can appreciate its current capabilities and potential future developments.
Principles of Electroformation
The principles of electroformation serve a crucial role in understanding how materials are formed at the molecular level through electrochemical processes. This section highlights the specific elements integral to electroformation, such as basic electrochemical processes and material interactions. These principles shape not only the methodology of electroformation but also its practical applications across myriad fields. Understanding these principles is essential for researchers and professionals who are exploring innovative ways to manipulate and utilize materials.
Basic Electrochemical Processes
Electrode Reactions
Electrode reactions form the foundation of electroformation. They involve the transfer of electrons between an electrode and the electrolyte, which is fundamental in creating new materials. The key characteristic of electrode reactions is their ability to drive the electrochemical reactions that lead to material formation. This property makes electrode reactions a popular choice for this article because they are at the core of the electroformation process.
A unique feature of electrode reactions is their direct influence on the microstructure of the resulting materials. Altering parameters such as current density and potential can significantly affect the properties of the resultant materials. The advantages of understanding electrode reactions include the capacity to optimize reactions for desired outcomes. However, there are disadvantages, such as the complexity of accurately controlling these reactions under various conditions.
Mass Transport Phenomena
Mass transport phenomena refer to how species move within the electrolyte during the electroformation process. This aspect is critical as it affects the uniformity and efficiency of material deposition. The key characteristic of mass transport phenomena is its direct impact on the rate of electrochemical reactions. By effectively managing mass transport, one can enhance the overall efficiency of electroformation, establishing its significance in this article.
A unique feature of mass transport phenomena is the interplay between diffusion and convection in electrolyte solutions. This affects how evenly materials can form across the electrode surface. The advantages of focusing on this aspect include improving deposit quality and rates. However, it can also introduce challenges, especially when maintaining uniform conditions across larger systems.
Material Interactions During Electroformation
Material interactions are pivotal during the electroformation process. This encompasses both charge transport and surface chemistry considerations, influencing how materials behave during formation.
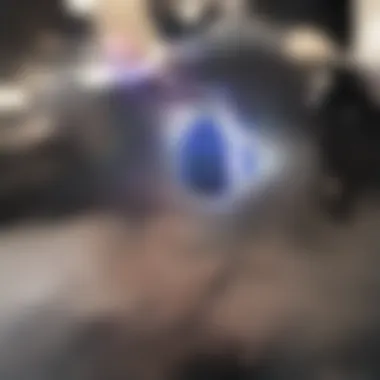
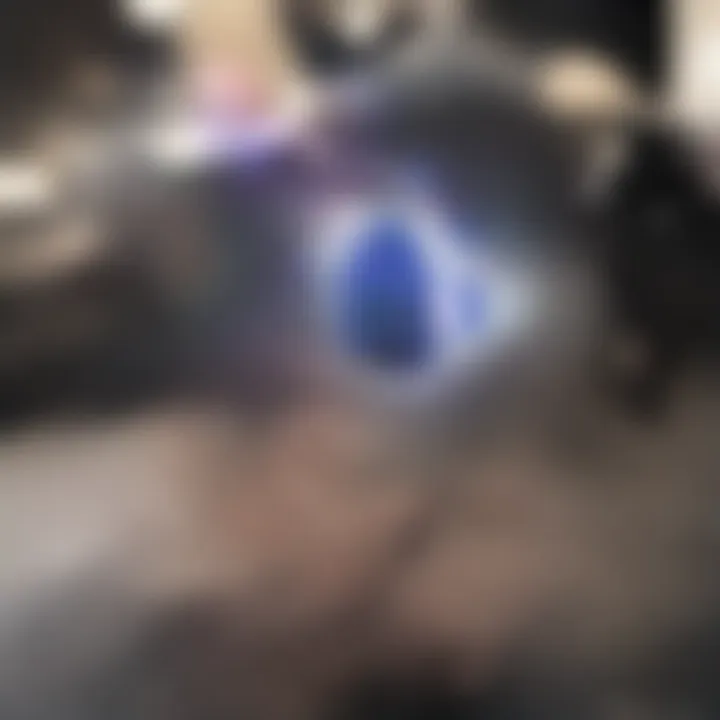
Charge Transport Mechanism
The charge transport mechanism is essential for facilitating the transport of ions to the electrode surface. This aspect is crucial because efficient charge transport ensures that electrochemical reactions proceed smoothly. The key characteristic of the charge transport mechanism is its influence on overall efficiency and material quality. This foundational aspect makes it a beneficial point of focus in this article.
A notable feature of charge transport mechanisms is their dependence on both ionic mobility and the concentration gradients in the electrolyte. This results in different behaviors based on the specific conditions applied during the process, impacting the final material properties. The advantages of optimizing charge transport include increased rates of deposition. However, the disadvantages often stem from dealing with complex ionic interactions that can challenge material consistency.
Surface Chemistry Considerations
Surface chemistry considers how materials interact at the molecular level during electroformation. This interaction is heavily impacted by factors such as surface energy and reactivity. A key characteristic of surface chemistry is its role in determining how deposits adhere and evolve during the electroformation process. This makes surface chemistry a vital aspect of this article.
A unique feature of surface chemistry considerations is their ability to dictate not just adhesion but also the nucleation and growth of new materials. Understanding these interactions can lead to enhanced material properties, such as conductivity or mechanical strength. However, the challenges often include the need for precise control over surface conditions to achieve desired results without unwanted side reactions.
Electroformation Techniques
Electroformation techniques are pivotal in the production of complex structures through the application of electrochemical processes. These techniques facilitate the manipulation of materials at the microscopic and nanoscopic levels, enabling the creation and transformation of various substrates and elements. The importance of understanding these techniques lies in their application across multiple fields, such as material science, biomedical engineering, and energy solutions.
Methods of Application
Direct vs. Indirect Electroformation
Direct electroformation involves applying electrical energy directly to the material that needs to be shaped or modified. This method allows for a straightforward approach, which is particularly useful in scenarios where fine control over the deposition process is paramount. The key characteristic of direct electroformation is its ability to cater to the specificity of the final product requirements. Such specificity makes it an advantageous choice when precision is required in creating functional layers or intricate structures.
On the other hand, indirect electroformation utilizes intermediary layers that facilitate the deposition process. This approach can enhance certain characteristics, like the adherence of layers to the substrate. The unique feature of this method revolves around its ability to create composite materials that combine different functionalities through layer-by-layer construction. However, this may also introduce complications regarding the compatibility of materials, leading to potential challenges in overall performance.
Batch vs. Continuous Processes
Batch processes are characterized by processing fixed amounts of materials in distinct batches. Such an approach allows for thorough control over the conditions throughout the electroformation, ensuring each batch meets the desired specifications. A beneficial aspect of batch processing is the ability to produce a variety of structures or results based on specific tasks, enhancing versatility.
In contrast, continuous processes are designed to allow an ongoing flow of materials through the electroformation system. This method can significantly increase production efficiency and reduce lead times, making it a popular choice in industrial applications. While this efficiency can have significant economic benefits, it may also lead to challenges regarding consistency and reproducibility across the produced materials. The unique challenge here is maintaining quality control while scaling up production.
Equipment and Setup
Electrolytic Cells
Electrolytic cells are crucial components in electroformation techniques. They provide the environment for the electrochemical reactions essential for material deposition and modification. A key characteristic of electrolytic cells is their ability to control various environmental parameters, like temperature and pH, thereby influencing the electrochemical processes significantly. They are beneficial to maintain the stability and uniformity of the deposition process.
Each electrolytic cell varies based on the intended processes and can be tailored to meet specific operational requirements. However, if not designed properly, there can be issues related to inefficiency and energy consumption in some setups. Therefore, careful consideration is essential during the selection and design phase of electrolytic cells to ensure optimal performance.
Controlling Parameters
Controlling parameters during electroformation is fundamental for achieving desired results. Critical factors include current density, temperature, and duration of exposure to the electrical field. The key characteristic lies in the necessity to maintain consistent and precise control over these parameters, as fluctuations can lead to variable outcomes.
Implementing advanced monitoring systems can facilitate real-time adjustments, enhancing reproducibility and product quality. While sophisticated monitoring does come at a cost, the benefits in terms of quality assurance and process optimization can justify the investment. Overall, meticulous control over parameters plays an essential role in the effectiveness of electroformation techniques, leading to better material properties and performance.
Materials Used in Electroformation
The choice of materials in electroformation is critical for achieving the desired outcomes in this unique fabrication process. Different materials exhibit distinct properties that influence their performance, stability, and applicability. Understanding the various characteristics of these materials is essential for optimizing the electroformation process and achieving high-quality results. In this section, we will examine the two primary categories of materials used in electroformation: conductive materials and insulating materials. Each type plays a unique role, contributing significantly to the effectiveness and applicability of the electroformation process.
Types of Materials
Conductive Materials
Conductive materials are fundamental in electroformation. Their primary function is to facilitate the passage of electrical current, which is necessary to initiate the electrochemical reactions that enable the formation of structures. A key characteristic of conductive materials is their high electrical conductivity, which allows for efficient charge transport during the electroformation process. Metals like copper and silver, as well as conductive polymers, belong to this category.
The benefit of using conductive materials lies in their ability to create robust and stable structures. For instance, metals possess excellent mechanical properties, which contribute to the overall durability of the formed materials. However, one significant disadvantage is their tendency to oxidize, which can affect the long-term performance of the electroformed products. Therefore, a careful selection of conductive materials is essential, ensuring that oxidation stability is maintained throughout the process.
Insulating Materials
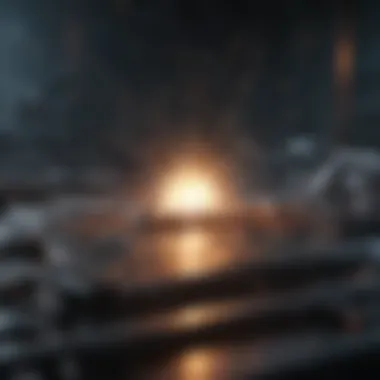
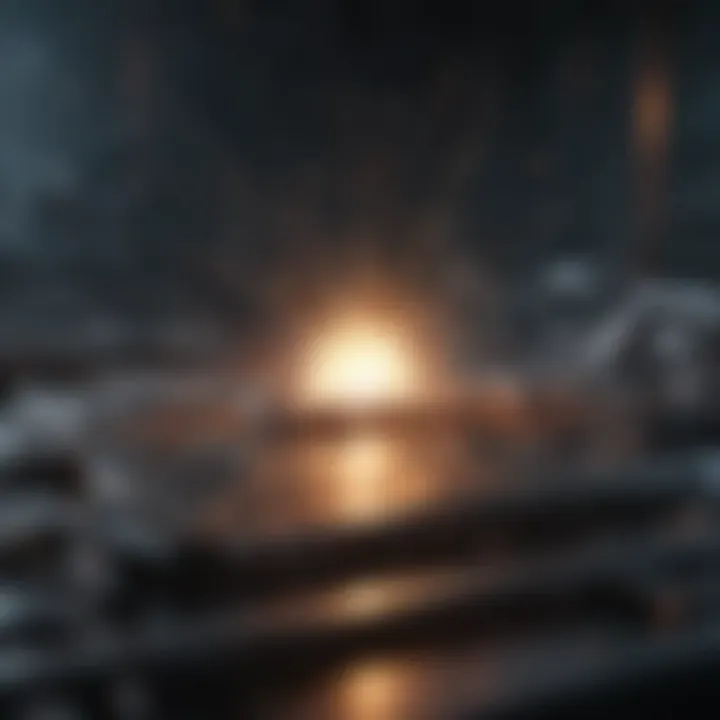
Insulating materials play a complementary role in electroformation. They are utilized primarily to control the electrical environment and prevent unwanted current paths that may lead to defects or undesired morphologies in the final products. The key characteristic of insulating materials is their low electrical conductivity, which helps to guide the electrochemical reactions in a controlled manner.
Insulating materials, such as certain polymers and ceramics, are considered beneficial in ensuring precision in the electroformation process. Their unique feature is the ability to maintain structural integrity under varying electrochemical conditions, thereby enhancing the overall quality of the resulting products. A potential downside is their limited conductivity, which may require careful synergy with conductive materials to ensure effective electrochemical activities.
Selection Criteria for Materials
When selecting materials for electroformation, several criteria must be considered to optimize the results:
- Electrical Properties: The conductivity and resistivity of the materials are crucial for efficient electroformation.
- Mechanical Stability: The structural integrity of materials under varying conditions is critical to maintain performance.
- Chemical Compatibility: Compatibility between materials is essential to avoid undesired reactions that can hinder the process.
- Cost Considerations: The economic aspect cannot be ignored, as the overall feasibility of the electroformation process is affected by material costs.
Applications of Electroformation
The applications of electroformation play a vital role in a variety of fields, emphasizing its significance in modern science and technology. This process is distinct for its ability to produce tailored materials that meet specific requirements. From nanotechnology to biomedical applications, the versatility of electroformation is evidenced by its wide-ranging uses and impact.
Nanotechnology and Material Science
Nanoscale Structures
Nanoscale structures are crucial in the realm of nanotechnology. They often enable enhanced properties not seen in bulk materials. A key characteristic of nanoscale structures is their high surface area-to-volume ratio, which can lead to improved reactivity and performance. In this context, electroformation is a beneficial method because it allows precise control over the morphology and dimensions of nanostructures.
One unique feature of these structures is their quantum effects, which can enhance their electronic properties. However, while they provide significant advantages in applications, it is crucial to consider the challenges of scalability and uniformity.
Composite Materials
Composite materials also find significant use in various domains. These materials combine two or more constituent materials to create a product with superior properties, such as improved strength and reduced weight. A key aspect of composite materials is their adaptability; they can be engineered to meet specific performance criteria, making them a popular choice in the industry.
The unique feature of composite materials is their ability to exhibit synergistic effects, where the combined performance surpasses that of individual components. In terms of disadvantages, there can be concerns regarding long-term durability and potential high costs associated with certain composites.
Biomedical Engineering
Tissue Engineering
Tissue engineering is an innovative area that greatly benefits from electroformation. It focuses on creating artificial organs or tissues that can integrate with human biology. A notable characteristic of tissue engineering is its potential to revolutionize transplant medicine, reducing dependency on donor organs. This is why it is seen as a significant advancement in health care.
The unique feature of tissue engineering is its ability to create scaffolds that support cell growth. While this presents great promise, challenges like biocompatibility and the replication of complex tissue structures must be addressed.
Drug Delivery Systems
Drug delivery systems are an essential application of electroformation in biomedical engineering. These systems are designed to deliver therapeutic agents in a controlled manner. A defining characteristic is their ability to target specific sites within the body, ensuring the drug takes effect where needed most. This focus enhances the efficiency of treatments.
The unique advantage of advanced drug delivery systems is the incorporation of nanotechnology to allow for real-time monitoring of drug release. However, creating effective systems that minimize side effects can be complex and requires ongoing research.
Energy Storage and Conversion
Battery Technologies
Battery technologies are vital in the quest for sustainable energy solutions. Electroformation can facilitate the development of advanced battery materials with improved energy density and longevity. One key aspect of battery technologies is their role in electric vehicles and renewable energy storage, making them a critical area of research.
One unique feature of electroformed battery components is the ability to fabricate thin films with uniform properties. However, high costs associated with advanced materials can be a barrier to wide-scale adoption.
Fuel Cells
Fuel cells are another promising application, converting chemical energy directly into electrical energy. The characteristic feature of fuel cells is their efficiency and lower emissions compared to traditional combustion engines. This makes fuel cell technology a compelling alternative in the pursuit of clean energy solutions.
The unique aspect of fuel cells lies in their ability to operate continuously as long as fuel is supplied. However, issues related to hydrogen storage and infrastructure development present significant challenges for widespread implementation.
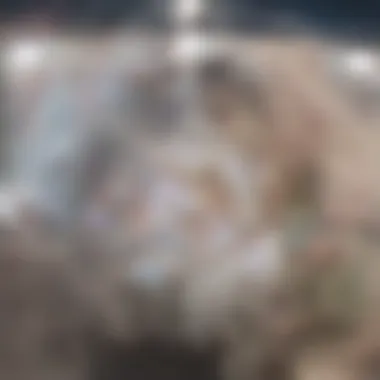
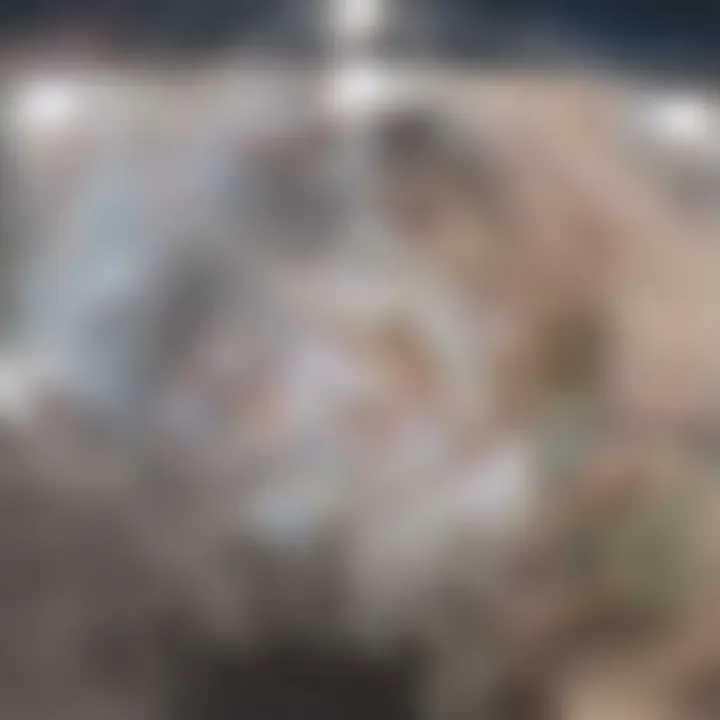
Electroformation offers innovative possibilities in fields ranging from nanotechnology to energy solutions, showcasing its broad potential impact in diverse applications.
Challenges in Electroformation
The electroformation process presents numerous obstacles that researchers and practitioners must navigate. These challenges affect the feasibility and quality of the final products. Understanding these issues is crucial for advancing the field and optimizing output across various applications, from energy storage to biomedical engineering.
Technical Limitations
Scalability Issues
Scalability is one of the more pressing concerns in electroformation. As demand for advanced materials grows, the methods used for electroformation must adapt to larger scales without compromising quality. The characteristic limiting factor is often the ability to maintain consistent results during the transition from laboratory to industrial scales. This aspect argues for the need of better process engineering.
A unique feature of scalability issues in electroformation is its dependency on the uniform distribution of electric fields across a larger surface. This non-uniformity can lead to inconsistencies in product characteristics like thickness and conductivity. Therefore, while exploring industrial applications, these factors can significantly impact performance and reliability.
Reproducibility Concerns
Reproducibility is a significant issue in electroformation processes. Variability in outcomes can stem from numerous factors, including differences in material properties and environmental conditions during formation. The central characteristic of reproducibility concerns is their ability to affect scientific studies and industrial applications. Without consistent results, credibility and effectiveness are at risk.
One unique aspect of reproducibility issues is how they relate to process parameters. Slight variations in temperature, current density, or electrolyte composition can lead to vastly different outcomes. This inconsistency can be disadvantageous, as researchers may find it difficult to replicate experiments, affecting further scientific validation
Material Constraints
Oxidation Stability
Oxidation stability is pivotal when considering materials for electroformation. Many conductive materials can suffer degradation in oxidative environments, impacting the longevity of the resultant structures. The main characteristic of this stability is its direct link to performance in practical applications. Materials that cannot maintain their properties under oxidative stress can lead to premature failure in devices such as batteries or other electronic components.
A unique feature to consider with oxidation stability is how it limits material selection. If a material has high conductivity but low oxidation stability, it may not be suitable for long-term applications. This presents a major disadvantage, leading researchers to find alternative solutions, which might also come with trade-offs.
Cost of Materials
The cost of materials is another significant constraint in electroformation. High-quality raw materials often lead to better performance, but the financial implications can limit their use, especially in commercial ventures. The key characteristic of material cost is its direct correlation with the scalability of electroformation processes.
Interestingly, the economic aspect of materials also ties into sustainability. Finding cost-effective materials that do not compromise performance is a challenge. The unique feature of the cost constraint involves material sourcing, which can influence the long-term sustainability of electroformation and affect projects at both academic and industrial levels.
"Understanding the intricacies of these challenges is essential for paving new frontiers in electroformation technology, enhancing its effectiveness across a variety of disciplines."
Addressing these challenges head-on can lead to substantial advancements in the field. By navigating the technical limitations and material constraints, the path toward improved electroformation methodologies can be forged.
Future Directions in Electroformation Research
The field of electroformation continues to grow, driven by advancements in technology and new material demands. Understanding the future of this process is critical for researchers and industries alike. As we explore innovative approaches, there are various opportunities for enhancement in energy applications, biomedical fields, and smart material integrations. By focusing on these directions, we not only highlight the relevance of electroformation but also address its potential benefits and challenges.
Innovative Approaches
Integration with Other Fabrication Techniques
Integrating electroformation with other fabrication techniques stands as a major trend in advanced manufacturing. Methods such as additive manufacturing and traditional lithography can elevate the capabilities of electroformation. This synergy allows for multi-layered structures with tailored properties. One key characteristic of this integration is the precision it offers in crafting complex geometries. This precision is advantageous, particularly in fields like nanotechnology where accuracy is paramount.
A unique feature of this approach is its adaptability. Combining it with existing methods can enhance the efficiency and scope of production. However, it's important to consider the potential pitfalls. The complexity of coupling techniques may introduce new variables that require careful management.
Smart Materials Development
The development of smart materials presents a fascinating avenue for electroformation. Smart materials are responsive to environmental stimuli. They can change properties based on temperature, light, or pressure. This characteristic makes them valuable in a variety of applications including sensors and actuators.
One key aspect of smart materials is their ability to enhance functionality without significantly increasing weight or bulk. This makes them especially appealing for applications in aerospace and medical devices, where space and weight are critical factors. A primary advantage is that they can lead to the creation of devices that self-regulate or self-heal, which is a significant step forward in technology.
However, challenges do exist. The integration of smart materials into existing systems may require extensive redesigns. This could increase costs and lead times, a consideration that cannot be overlooked when implementing new technologies.
Potential for Interdisciplinary Applications
The future landscape of electroformation also promises interdisciplinary applications that span across different fields. From collaborations between material scientists and biomedical engineers to partnerships with energy specialists, the potential for innovation is vast.
This interdisciplinary approach fosters diverse problem-solving techniques and encourages the development of novel solutions. For instance, combining materials science with electrical engineering can lead to improved energy storage systems. In medicine, synergy with biological sciences may result in advanced drug delivery mechanisms that improve patient outcomes.