Exploring the Depths of Optical Fluorescence Microscopy
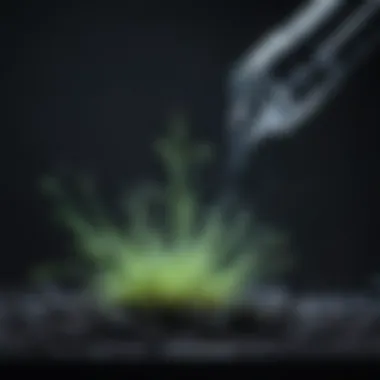
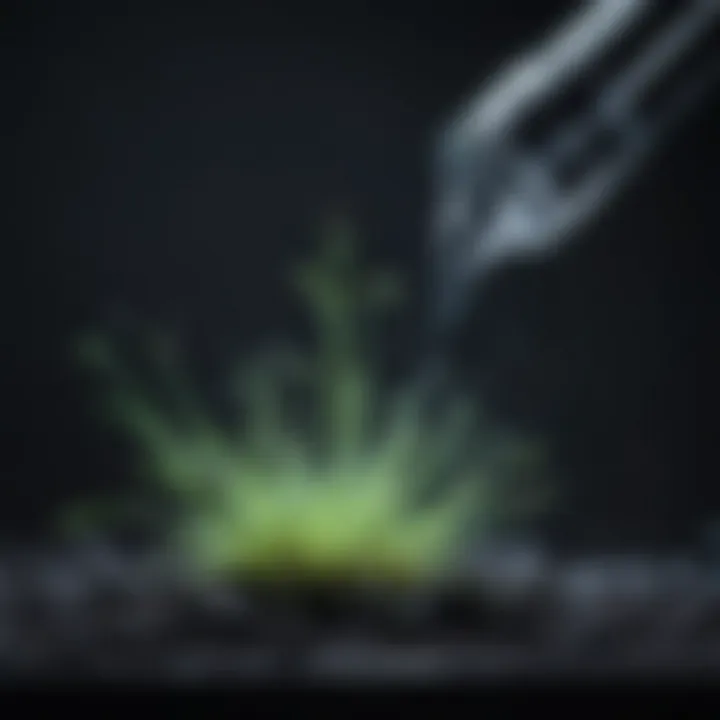
Intro
Optical fluorescence microscopy stands at the forefront of modern scientific imaging techniques. Employed across a variety of fields, it allows researchers to peer into the intricate details of cellular and molecular processes in ways that traditional microscopy simply cannot achieve. The essence of this technology lies in its ability to exploit the unique properties of fluorescence, enabling the visualization of specific components within a sample. As such, it has become a cornerstone in biological research, materials science, and even clinical diagnostics.
The brilliance of fluorescence microscopy is not just in its definition but in the astonishing advancements that have propelled it forward. From the more traditional methods using basic fluorescence to cutting-edge techniques such as super-resolution microscopy, the evolution has been remarkable. Researchers are now equipped with tools that sharpen images at the nano-scale, providing insights that were previously hidden in the shadows.
In exploring the utility of optical fluorescence microscopy, one cannot overlook its diverse range of applications. This technique has revolutionized how scientists understand cellular mechanisms, track molecular interactions, and even assess the efficacy of new drugs. Thus, gaining an in-depth understanding of how it works, what innovations are on the horizon, and what challenges remain is essential for both newcomers and established practitioners.
This article endeavors to elucidate all these aspects, weaving together the principles, methodologies, and future directions of optical fluorescence microscopy into a cohesive narrative that should resonate with the scientific community. Whether you are a student eager to learn the fundamentals or a seasoned researcher looking to refine your knowledge, this exploration offers a path to greater understanding.
Key Findings
Major Results
The significance of optical fluorescence microscopy can be summarized in several key findings:
- High-resolution imaging capabilities that surpass traditional microscopy techniques.
- Enhanced specificity in detecting cellular components through targeted fluorescence.
- Ability to observe live cell dynamics at unprecedented temporal resolution.
- Revolutionary applications in drug discovery and understanding complex biological systems.
"Optical fluorescence microscopy has opened new windows into the microscopic world, allowing for an unparalleled view of dynamic processes in real-time."
Discussion of Findings
These findings underscore the importance of fluorescence microscopy in research environments. The ability to visualize live processes in real time enables scientists to gather data that were previously unattainable. For instance, the development of fluorescently labeled antibodies has made it possible to identify and track multiple cellular targets simultaneously. This multicolor capability enhances our understanding of cellular interactions and signals, painting a more comprehensive picture of cellular behavior.
Methodology
Research Design
The methodologies employed in fluorescence microscopy have undergone significant refinement. Researchers typically adopt a multi-faceted approach, combining various imaging techniques and optical components that augment the capabilities of standard fluorescence microscopes. The design strategy often involves integrating lasers for excitation, sophisticated filters for wavelength separation, and advanced detectors that provide clarity and precision in quantifying fluorescence emission.
Data Collection Methods
Data collection in optical fluorescence microscopy can be done through various methods:
- Live-cell imaging: This method allows for observing dynamic processes in real-time, providing insights into cellular behaviors over time.
- Fixed-cell imaging: Samples are treated and fixed, allowing researchers to examine specific interactions at a moment in time.
- Time-lapse microscopy: Capturing images over time to study the effects of treatments or environmental changes on cells can unveil critical patterns and dynamics.
Through these methodological lenses, the article will explore the remarkable capabilities of optical fluorescence microscopy, illustrating how it has transformed research methodologies across disciplines. Such an exploration is key not just to appreciating the current state of the field but also for contemplating future innovations.
Preface to Optical Fluorescence Microscopy
Optical fluorescence microscopy stands as a prominent technique in modern science, bridging the gap between molecular biology and materials science. It provides unparalleled detail and contrast, enabling researchers to visualize structures that were once mere abstractions on a theoretical level. This method not only enhances our understanding of cellular mechanisms but also empowers advancements across various fields, including neuroscience and environmental studies.
Definition and Significance
Optical fluorescence microscopy can be defined as an imaging technique that uses fluorescent light to examine biological and material specimens. This process involves exciting fluorescent molecules, known as fluorophores, with a specific wavelength of light, prompting them to emit light at a different wavelength. The result is a vivid and detailed image that highlights even the most intricate cellular components.
Fluorescence microscopy eliminates the need for complex staining procedures typically associated with traditional microscopy, thus preserving the integrity of live specimens. With its ability to capture dynamic processes in real time, this technique has become essential for tracking molecular interactions, monitoring cellular behaviors, and assessing drug efficacy in live cells.
"In the context of modern research, the precision offered by optical fluorescence microscopy is nothing short of revolutionary."
Historical Context
The journey of optical fluorescence microscopy can be traced back to the early 20th century, when scientists began experimenting with the principles of fluorescence. The groundwork laid by pioneers such as Wilhelm Conrad Röntgen, who discovered X-rays, and Max Knoll, who contributed to the development of electron microscopy, paved the way for the fluorescence revolution.
It wasn’t until the 1960s, however, that the first commercial fluorescence microscopes emerged, allowing researchers to exploit this novel technique in their work. The development of highly sensitive detectors and advanced light sources further propelled this technique into the spotlight.
As fluorescence microscopy evolved, so did the range of applications. From essential studies in cell biology to breakthroughs in medical diagnostics, the significance of this technique has grown immensely. Today, fluorescence microscopy has not only transformed how we view biological systems but also paved the way for interdisciplinary investigations, intertwining biology with physics, chemistry, and engineering, leading to unprecedented insights in scientific research.
Fundamental Principles of Fluorescence
Fluorescence microscopy has transformed the landscape of biological and materials research, allowing scientists to visualize complex molecular interactions with remarkable clarity. At the core of this imaging technique lies the fundamental principles of fluorescence, which not only drive the technology behind the microscopes but also influence their application across various scientific fields. Understanding these principles sets the stage for grasping how fluorescence can be harnessed effectively in research environments.
Mechanism of Fluorescence
The mechanism underlying fluorescence involves the absorption of light by a substance, followed by the re-emission of light at a longer wavelength. This is due to the excitation of electrons within the atoms of a molecule, elevating them to a higher energy state. Once the electrons return to their resting state, they release energy in the form of light. This luminescence phenomenon is not merely serendipitous; it’s a well-orchestrated symphony of atomic interactions.
To illustrate: consider a sunflower in full bloom. When sunlight strikes the flower, its vibrant yellow petals absorb certain wavelengths of light and reflect others. Similarly, fluorescent molecules absorb specific light waves and then emit distinct colors, which can vary based on the molecule’s structure. This is significant in fluorescence microscopy as it allows researchers to differentiate between various cellular components, revealing intricate details of biological processes.
Types of Fluorophores
Fluorophores play a crucial role in fluorescence microscopy; they are the molecules responsible for the fluorescence effect. There’s a diverse array of fluorophores used in various applications, each chosen depending on their spectral properties and how they interact with biological samples.
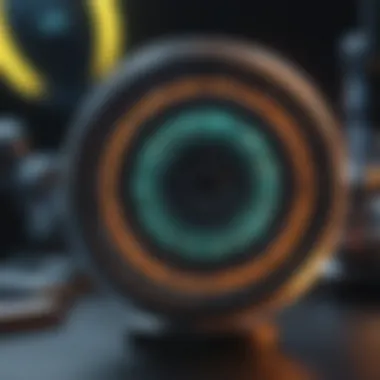
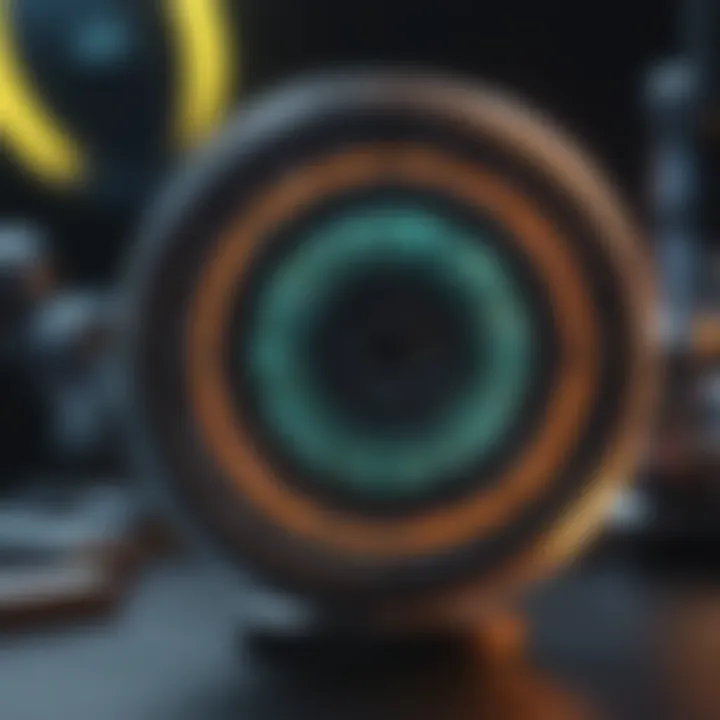
- Dyes: Commonly used in cellular imaging. Dyes like fluorescein and rhodamine are examples. They are relatively inexpensive and provide a range of fluorescent characteristics that aid in studies of complex biological systems.
- Proteins: Green fluorescent protein (GFP) has become a standard in molecular biology due to its ability to tag proteins in living cells without the need for additional substrates. Their versatility has launched innovative techniques, such as live-cell imaging.
- Quantum Dots: These nanometer-scale semiconductor particles exhibit tailored optical properties and are particularly useful in multiplexing applications due to their broad absorption spectra and narrow emission bands.
Choosing the right fluorophore is not just about the terms of brightness and stability; factors like photobleaching potential and toxicity can significantly impact experimental outcomes. Hence, understanding these options is essential for effective imaging.
Excitation and Emission Processes
The excitation and emission processes of fluorescence are fundamental to understanding how fluorescent microscopy operates. Excitation typically begins with a light source, like a mercury arc lamp or laser, that emits light at specific wavelengths tailored to excite the fluorophores in the sample.
After excitation, the excited electrons swiftly return to their ground state, emitting photons in the process. This emitted light is collected and analyzed to create an image, the quality and detail of which hinge on the efficiency of both the excitation and emission processes.
Key Considerations:
- Light Source: Proper selection of light sources ensures maximal excitation of fluorophores.
- Filters and Dichroic Beamsplitters: These components are essential; they control the wavelengths of light that reach the detectors, allowing for clean separation of excitation and emission light.
- Environment: The surrounding conditions, like temperature and pH, can influence the fluorescence yield and overall imaging quality.
"Fluorescence spectroscopy allows for real-time observation of cellular dynamics, fostering a profound understanding of biological processes."
In summary, a firm grasp of fluorescence mechanisms, the variety of fluorophores available, and the excitation-emission processes is vital for anyone looking to leverage optical fluorescence microscopy in their work. As technology progresses and new agents are discovered, the potential applications for fluorescence microscopy continue to expand, promising to illuminate even more of the world at the molecular level.
Key Components of Optical Fluorescence Microscopes
Optical fluorescence microscopes are remarkable tools, fundamentally relying on specific components that work harmoniously to produce high-resolution images of fluorescent samples. Understanding these components is crucial for anyone engaged in or studying this field, as they play a significant role in imaging quality and experimental outcomes. The key components include the optical system, filters, dichroic beamsplitters, detectors, and imaging sensors. Each of these elements contributes uniquely to the performance of the microscope and enables the visualization of intricate biological and material structures that might otherwise remain unseen.
Optical System Overview
The optical system is the backbone of any fluorescence microscope. It consists of a series of lenses that manipulate light to focus on the specimen. This arrangement typically includes an objective lens, which has a significant impact on the resolution and magnification of the images captured. The quality of the optics can vary, and hence, microscope manufacturers often aim for precision in lens construction and coatings.
In practical terms, a microscope’s optical system determines how well it can differentiate between closely positioned fluorescent structures. For instance, a higher numerical aperture on the objective lens allows for better light collection and sharper images, enhancing the visibility of fine details within the sample. It is like having a quality camera lens—better lenses yield better pictures.
Filters and Dichroic Beamsplitters
Filters and dichroic beamsplitters are essential for selecting specific wavelengths of light during fluorescence microscopy. These components are crucial for isolating the desired excitation light while allowing the emitted fluorescence to pass through.
- Excitation Filters: These filters block wavelengths that could interfere with imaging while letting only the appropriate wavelengths through, stimulating the fluorophores in the specimen.
- Dichroic Beamsplitters: Positioned within the optical pathway, dichroic beamsplitters reflect specific wavelengths of light, directing the excitation light toward the specimen while allowing emitted light to pass through toward the detector.
Setting up the right combination of filters and beamsplitters can take some finesse; it’s a bit like tuning a musical instrument for the best performance. The quality of these components not only affects the brightness of the images but also their contrast, making them vital in ensuring high-quality microscopic images.
Detectors and Imaging Sensors
The final analysis of fluorescence microscopy hinges significantly on detectors and imaging sensors. They play the essential role of capturing the emitted light from the sample and converting it into a readable signal. Detectors can vary depending on the applications and may include photomultiplier tubes (PMTs) or charge-coupled devices (CCDs).
- Photomultiplier Tubes (PMTs): These are extremely sensitive devices, ideal for low-light applications often encountered in fluorescence microscopy. They can amplify the weak fluorescent signals from the sample, making them crucial for capturing faint emissions.
- Charge-Coupled Devices (CCDs): These sensors are often favored for their ability to capture high-resolution images with low noise levels. They excel in situations where detailed observations are needed and are more commonly used in digital microscopy setups.
The choice of detector can greatly influence the resulting image quality and the effectiveness of the analysis. An accurate and efficient imaging sensor leads to better interpretation of experimental data, enhancing the reliability of the findings.
Imaging Techniques in Fluorescence Microscopy
Imaging techniques are at the heart of optical fluorescence microscopy. The variety of methods available caters to different research needs and allows for the visualization of biological structures at various resolutions and depths. Each technique offers unique advantages and considerations that can significantly affect outcomes in scientific studies.
Widefield Fluorescence Microscopy
Widefield fluorescence microscopy provides a straightforward imaging solution for observing samples. This method utilizes a coherent light source, such as a mercury or xenon lamp, to excite fluorophores across the entire field of view simultaneously. Thus, widefield is often celebrated for its simplicity and speed, allowing researchers to quickly observe large samples or screen multiple conditions in a short time frame.
However, this technique can suffer from notable challenges. One significant issue is the problem of out-of-focus light. Because it illuminates the entire area, any varying depths within the sample lead to blurring in the final images. To mitigate this, careful adjustments in image acquisition and processing are necessary.
"In widefield microscopy, sampling many signals at once can lead to noise, yet it remains a cornerstone for quick surveys of specimen."
Confocal Microscopy
Confocal microscopy leaps forward in the quest for clarity by employing a focused laser beam that scans across the sample. It selectively illuminates only a thin slice at any given moment. This approach minimizes out-of-focus light and creates images of significantly enhanced resolution and contrast compared to widefield techniques.
One of the noteworthy features of confocal microscopy is its ability to produce 3D reconstructions of samples. By gathering images at different depths, researchers can compile a comprehensive view that encapsulates complex spatial relationships within the sample. However, this advanced imaging may take longer than widefield methods, required more time to gather and process multiple z-planes.
Challenges also exist, particularly regarding photobleaching—a phenomenon where fluorophores lose their ability to fluoresce as they are exposed to radiation—affecting quantitative analysis.
Total Internal Reflection Fluorescence (TIRF) Microscopy
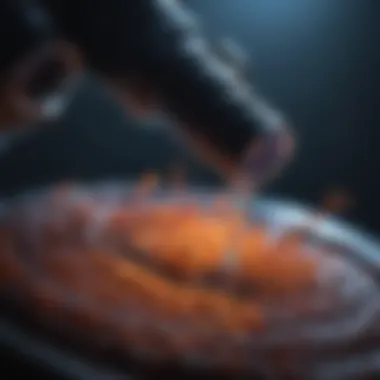
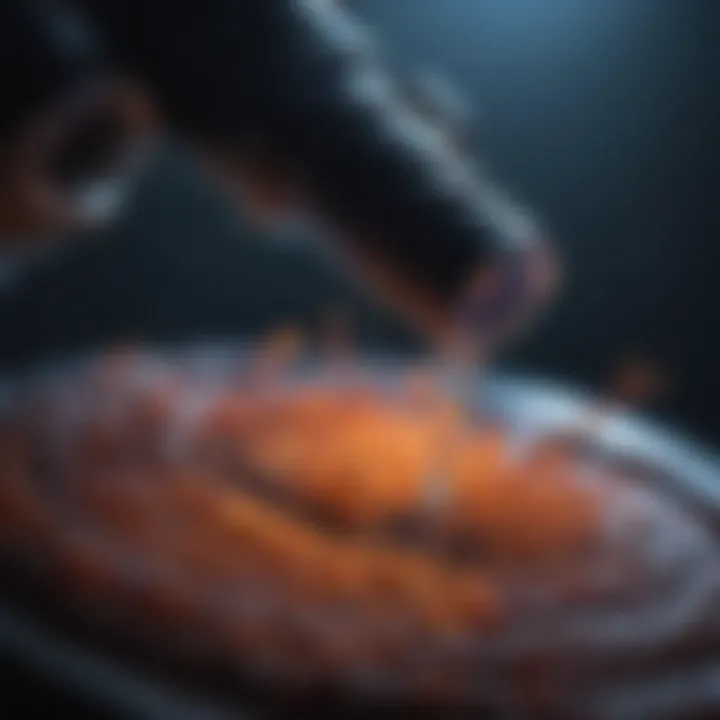
Total Internal Reflection Fluorescence microscopy is a specialized imaging technique that shines light at a specific angle, causing an evanescent wave to excite fluorophores near the surface of a slide or coverslip. This technique is particularly suited for studying processes that occur at or near the plasma membrane of cells. The dramatic drop-off in excitation intensity beyond the surface allows for single-molecule imaging and the study of dynamic processes like membrane trafficking.
TIRF delivers images with exquisite clarity, yet it comes with its own set of limitations. Primarily, the excitation is restricted to a very thin region of the sample, making it less ideal for samples requiring volumetric imaging. Furthermore, careful sample preparation and alignment are essential for optimal results.
Fluorescence Lifetime Imaging Microscopy (FLIM)
Fluorescence Lifetime Imaging Microscopy represents a nuanced approach to fluorescence imaging by focusing on the time that fluorophores remain in an excited state before emitting a photon. This measurement can inform scientists about the local environment, interactions between molecules, and even cellular pathways.
FLIM is powerful in dissecting complex biological processes and can pinpoint changes in molecular interactions that occur in real time. Despite its advantages, implementing FLIM requires sophisticated equipment and analysis tools, which may limit its accessibility for some labs.
In summary, each imaging technique in fluorescence microscopy serves a distinct purpose and has its strengths and weaknesses. From the rapid surveys in widefield microscopy to the high-resolution capabilities of confocal microscopy and the specialized usages of TIRF and FLIM, understanding these methodologies equips researchers to choose the most appropriate technique for their specific needs.
Applications of Optical Fluorescence Microscopy
Optical fluorescence microscopy has carved out a vital role in various scientific disciplines, acting as a keystone for advanced imaging techniques. The significance of this technology transcends mere visualization; it empowers scientists to glean insights into cellular processes, structural dynamics, and molecular interactions with remarkable clarity. By illuminating biological specimens and materials at the microscopic level, this technique not only elucidates fundamental biological mechanisms but also evaluates material properties critical in various fields.
Cell Biology and Molecular Imaging
Cell biology thrives on the rich data provided by fluorescence microscopy. This application allows researchers to visualize distinct cellular components in real time. For instance, understanding cellular processes such as signal transduction, gene expression, and protein interactions has been revolutionized. The use of specific fluorophores designed to target particular organelles enables the precise imaging of structures like mitochondria or the nucleus, revealing critical insights relevant to cell health and disease.
For example, the targeted use of Green Fluorescent Protein (GFP) has made it possible to observe live cell dynamics, which allows scientists to trail protein interactions as they happen. This is crucial for mapping out pathways in cancer research, where alterations in cellular function can point to potential therapeutic targets. The ability to observe so intimately grants unprecedented insight that is invaluable for both academic and clinical contexts.
Neuroscience and Brain Imaging
In the realm of neuroscience, fluorescence microscopy serves as an essential tool for visualizing intricate brain structures and neuronal connectivity. By using various labeling techniques, researchers can track neuronal activity and connectivity in living organisms. The advent of calcium-sensitive fluorescent probes, for instance, has made it feasible to monitor real-time neuronal firing in response to stimuli, thus providing a window into brain function and dysfunction.
"Fluorescence microscopy in neuroscience has opened new avenues for understanding the brain's wiring and function."
Moreover, investigating neurodegenerative diseases through fluorescence imaging allows a detailed perspective on how pathological changes unfold at a cellular level. The organization changes, synaptic degeneration, and protein aggregation are features that can be captured with precision, yielding potential biomarkers for early diagnosis and therapeutic intervention.
Materials Science Applications
The applications extend beyond biology into materials science, where fluorescence microscopy aids in the characterization of new materials and nanostructures. The capability to visualize materials under specific conditions or in the presence of stimuli introduces powerful tools for understanding the properties of polymers, nanocomposites, and other advanced materials.
In materials science, the benefit of fluorescence imaging lies in its ability to reveal surface defects, distribution of additives, or even the phase separation in complex systems. This capability is instrumental for quality control and in finding applications, such as in the development of new electronic devices where material properties directly dictate performance. Monitoring how materials behave under stress or environmental changes can guide engineers in design processes, ultimately fostering innovations.
Clinical Diagnostics and Pathology
Finally, the application of optical fluorescence microscopy in clinical diagnostics cannot be overstated. Its capacity to enhance pathology through precise detection of anomalies in tissue samples underlies many diagnostic protocols today. By utilizing specific fluorochromes for certain markers, pathologists can differentiate between healthy and diseased tissues with greater accuracy.
For instance, fluorescence-guided surgery is an emerging field where surgeons can visualize malignant tissues in real time during operative procedures, significantly increasing the likelihood of complete tumor resection. Such advancements in diagnostics not only improve patient outcomes but also advance the field by providing clearer definitions of disease states through molecular signatures.
In summary, the applications of optical fluorescence microscopy are far-reaching and diverse. They play a crucial role not only in advancing scientific inquiry but also in practical applications that touch everyday life, from medical diagnostics to the development of innovative materials. It underpins our pursuit of deeper knowledge, precision, and efficacy across fields, ensuring its relevance as technology continues to evolve.
Advantages and Limitations
Optical fluorescence microscopy stands as a pivotal tool in the scientific toolkit, enabling researchers to visualize biological and material specimens at the cellular and molecular levels. However, like any advanced technique, it comes with its own set of advantages and limitations that warrant thorough exploration. Understanding these elements is crucial for effectively utilizing this technology in research and diagnostics.
Strengths of Fluorescence Microscopy
One of the most significant advantages of fluorescence microscopy is its ability to provide high sensitivity in detecting low-abundance biomarkers in complex samples. This characteristic allows scientists to study cellular processes in real-time and in a living context. For example, researchers can employ GFP-tagged proteins to investigate protein interactions and dynamics within live cells, revealing intricate biological phenomena that would otherwise remain hidden.
Additionally, the capacity to differentiate between multiple fluorophores is a notable strength. By using various fluorescent tags, one can visualize multiple targets simultaneously, offering insights into co-localization and cellular interactions.
- High Resolution: Fluorescence microscopy can achieve resolutions that surpass those of standard light microscopy due to its precise optical filters and detectors.
- Versatility: The technique can be utilized across a variety of fields, including biology, materials science, and medicine, making it broadly applicable.
- Photostability: Improved fluorophores now exhibit increased photostability, allowing extended imaging sessions without loss of intensity or clarity.
"The capacity of fluorescence microscopy to provide spatial and temporal information about cellular events is unmatched in many research settings."
Challenges and Limitations
Despite its many advantages, fluorescence microscopy is not without challenges. One primary limitation lies in photobleaching. Fluorophores, especially those that are less photostable, degrade when exposed to excitation light over prolonged periods. This can be particularly challenging during long-term imaging experiments, as it might lead to a loss of signal and inaccurate interpretations.
Moreover, the need for specific fluorescent tags imposes constraints on experimental design. Researchers must often engineer or obtain fluorophores that are compatible with their biological targets, which can complicate studies.
- Background Fluorescence: Non-specific signals from tissue or other cellular components can obscure the desired fluorescence, making quantitative analysis tricky.
- Complexity of Analysis: The interpretation of fluorescence imaging data requires a solid understanding of photophysics and image processing techniques, which may pose a steep learning curve for newcomers.
- Limited Depth Penetration: While fluorescence microscopy excels at surface imaging, its effectiveness diminishes when imaging deeper tissues due to scattering and absorption of light.
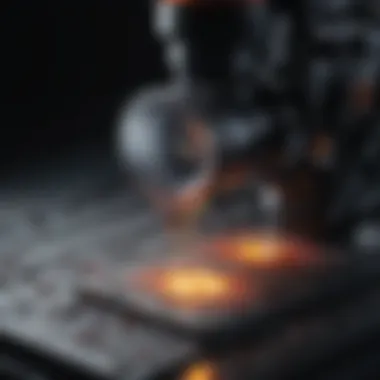
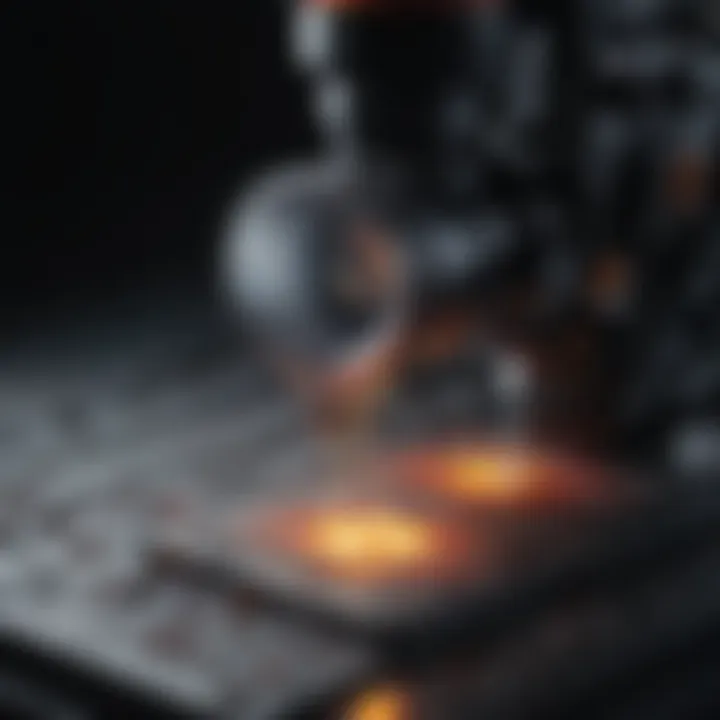
Future Directions in Optical Fluorescence Microscopy
The realm of optical fluorescence microscopy is ever-evolving, with advancements paving the way for refined techniques and broader applications. As environments for microscopic analysis become increasingly complex, the need for innovative approaches is apparent. The future possibilities in this field hold tremendous potential, making it critical to understand how these developments can shape scientific discovery and diagnostic practices.
Technological Innovations
Recent technological strides in optical fluorescence microscopy have set the stage for groundbreaking capabilities. For instance, the emergence of super-resolution microscopy techniques, like STORM (Stochastic Optical Reconstruction Microscopy), enables researchers to visualize cellular structures at a single-molecule level. This sharp level of detail is akin to using a magnifying glass on a tiny map—it reveals features that were simply invisible with traditional imaging methods. Such advancements enhance the accuracy of data collected, allowing scientists to make better-informed conclusions about complex biological processes.
Moreover, developments in multiphoton excitation techniques are enabling deeper tissue imaging without causing significant damage to the samples. The implications of these techniques are enormous within fields like neuroscience, where studying live brain tissue becomes feasible.
Potential for Integration with Other Techniques
The integration of optical fluorescence microscopy with other imaging modalities presents another promising frontier. For instance, coupling fluorescence microscopy with techniques like electron microscopy can provide complementary strengths. While fluorescence techniques offer insight into dynamic processes at the cellular level, electron microscopy provides exquisite detail of morphology at nanometer resolution. This synergy not only enhances the detail but also allows for more comprehensive data interpretation.
Additionally, the use of artificial intelligence in image analysis is rapidly gaining traction. Deep learning algorithms can process vast amounts of fluorescence images quickly. This results in refined data analysis and improved identification of patterns or anomalies in cellular behavior, rejuvenating the way researchers scrutinize their findings. It’s as though researchers are given a well-trained guide to navigate the vast landscape of microscopic data.
Expanding Applications in Various Fields
The applications of optical fluorescence microscopy are expanding beyond traditional biology and medicine into a multitude of disciplines. In the world of materials science, for example, fluorescence techniques are utilized to characterize nanomaterials, facilitating breakthroughs in technology and engineering. Understanding the interactions at a molecular level can lead to design innovations that catapult industries forward.
Furthermore, applications in environmental science are gaining traction, particularly in monitoring pollutants. The ability to tag and visualize specific contaminants in real-time allows for rapid responses to environmental hazards, making a significant impact on public safety.
In medicine, emerging techniques like fluorescence-guided surgery are enhancing the precision of operations. Surgeons can use real-time images provided by fluorescence staining methods to differentiate between healthy and diseased tissue more effectively, improving outcomes for patients.
As we continue to traverse these exciting pathways in fluorescence microscopy, the collaborative efforts across disciplines will undoubtedly fuel further advancements. The future is not just bright—it’s vividly illuminated with new possibilities.
Ethics and Safety in Fluorescence Microscopy
Ensuring ethical practices and safety is paramount in fluorescence microscopy, as these elements directly influence the reliability of results and the well-being of researchers. Investigators involved in this field work with various biological materials and chemical agents that can pose risks if not handled appropriately. Thus, addressing ethics and safety isn't just a matter of compliance; it’s a responsibility that underpins the very foundation of scientific inquiry.
Handling Fluorophores and Chemical Reagents
Fluorophores, the compounds that emit fluorescence upon excitation, are central to fluorescence microscopy. These substances are often chosen for their brightness and stability; however, many can be toxic or hazardous when mishandled. Researchers must familiarize themselves with the specific properties and risks associated with different fluorophores.
Some important considerations include:
- Material Safety Data Sheets (MSDS): Always refer to the MSDS for each fluorophore to understand its toxicological profile and recommended handling procedures.
- Personal Protective Equipment (PPE): Wearing appropriate PPE such as gloves, goggles, and lab coats is non-negotiable. These measures safeguard against exposure and ensure a secure working environment.
- Disposal Practices: Proper disposal protocols for chemical wastes should be followed. Fluorophores and other reagents often require specific disposal methods to minimize environmental impact.
Moreover, the ethical implications of using specific biological materials must be considered. Informed consent and appropriate permits should govern the use of tissues or cells, reflecting respect for ethical boundaries.
Safety Protocols in Microscopy Labs
A robust safety culture in microscopy labs is vital for promoting a safe working environment. Implementing stringent safety protocols can prevent accidents and mitigate risks associated with the use of advanced imaging techniques. Some key protocols include:
- Regular Training: Continuous education on laboratory safety practices keeps researchers informed about the latest safety measures and refreshes their memory on established practices.
- Emergency Procedures: Clearly defined emergency protocols should be in place, including access to safety showers, eyewash stations, and first aid kits. It’s crucial that all personnel know these procedures well.
- Routine Equipment Checks: Regular inspections and maintenance of microscopy equipment ensure that any potential hazards such as laser exposure or electrical malfunctions are addressed before use.
Incorporating a culture of open communication among lab members about safety concerns fosters an environment supportive of ethical behavior and proactive risk management.
“Safety doesn't happen by accident; it requires thoughtful practices and accountability from every member of the team.”
By emphasizing the significance of ethics and safety in fluorescence microscopy, researchers can uphold the integrity of their work, ensuring that they contribute positively to the scientific community.
Adhering to these ethical guidelines and safety protocols ultimately translates to greater trust in the findings of fluorescence microscopy, fostering continued innovation in research.
Finale
The conclusion of an article covering optical fluorescence microscopy holds significant weight in tying together the various components discussed. It acts as a wrap-up, crystallizing the dense information and offering readers a clear perspective on the topic. In an era where scientific visualization is paramount, understanding fluorescence microscopy's role becomes essential for students, researchers, and clinicians alike.
Summary of Key Insights
In summarizing the content, we underline several core insights:
- Visual Clarity: Optical fluorescence microscopy offers high-resolution images that can reveal cellular structures and dynamics that would be otherwise indiscernible by conventional methods.
- Diverse Applications: This technique shows its value across diverse fields, from biology and neuroscience to materials science, demonstrating its versatility.
- Ongoing Innovations: Continuous improvements in technology and methodologies unveil new horizons for more precise imaging techniques.
- Ethical Considerations: The necessity of adhering to safety protocols and ethical standards is paramount when dealing with the reagents and samples involved in fluorescence microscopy.
This holistic comprehension not only equips readers with knowledge but also prepares them to utilize these insights in practical settings, where these techniques can drive impactful discoveries.
The Future of Microscopic Imaging
Looking ahead, the landscape of microscopic imaging via optical fluorescence is poised for transformation. Several considerations emerge as we ponder the future:
- Integration with AI: Artificial intelligence is making inroads into image analysis, promising to enhance the interpretation of data at an unprecedented pace.
- Multi-modality Approaches: Combining fluorescence microscopy with other imaging modalities can yield comprehensive views of biological processes, allowing for greater understanding and exploration of interactions at multiple scales.
- Expanding into Clinical Settings: Adoption in clinical settings could revolutionize diagnostics, leading to more rapid and accurate identification of pathologies based on fluorescent markers.
- Emerging Fluorescent Probes: The development of novel fluorophores and improved labeling techniques is critical. Their capability to be used under various conditions will expand experiment versatility.
As we journey into the future, the advancements in optical fluorescence microscopy promise to sustain an edge in imaging technology. Future research and development in this field will not only enhance existing tools but will likely lead to entirely new approaches in science and medicine.